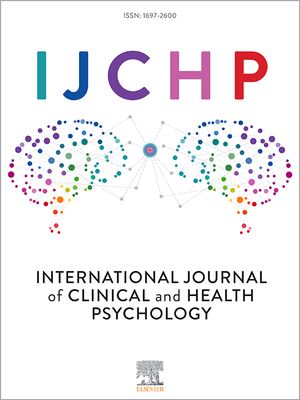
Edited by: Andre R Brunoni, Marie-Anne Vanderhasselt, Leigh Chavert
More infoAccumulating evidence shows that the posterior cerebellum is involved in mentalizing inferences of social events by detecting sequence information in these events, and building and updating internal models of these sequences. By applying anodal and sham cerebellar transcranial direct current stimulation (tDCS) on the posteromedial cerebellum of healthy participants, and using a serial reaction time (SRT) task paradigm, the current study examined the causal involvement of the cerebellum in implicitly learning sequences of social beliefs of others (Belief SRT) and non-social colored shapes (Cognitive SRT). Apart from the social or cognitive domain differences, both tasks were structurally identical. Results of anodal stimulation (i.e., 2 mA for 20 min) during the social Belief SRT task, did not show significant improvement in reaction times, however it did reveal generally faster responses for the Cognitive SRT task. This improved performance could also be observed after the cessation of stimulation after 30 min, and up to one week later. Our findings suggest a general positive effect of anodal cerebellar tDCS on implicit non-social Cognitive sequence learning, supporting a causal role of the cerebellum in this learning process. We speculate that the lack of tDCS modulation of the social Belief SRT task is due to the familiar and overlearned nature of attributing social beliefs, suggesting that easy and automatized tasks leave little room for improvement through tDCS.
The cerebellum has traditionally been considered as a uniquely motor controller, helping people learn automatized motor skills by building internal models of sequenced movements (Ito, 2008). However, emerging studies have revealed that cerebellar areas also play a critical role in mental, non-motor, processes (Ito, 2008; Van Overwalle et al., 2014). Large scale meta-analyses of fMRI studies with healthy participants revealed robust cerebellar involvement in cognitive functions, such as attention, working memory, executive control, and learning sequences (Guell et al., 2018; Schmahmann et al., 2019; Van Overwalle et al., 2014). More recently, neuroimaging studies have shown that the cerebellum also contributes to the social domain, such as mentalizing, which is the process of understanding others’ metal states (Ito, 2008; Metoki et al., 2021a; Premack & Woodruff, 1978; Van Overwalle et al., 2014). Meta-analyses of fMRI studies also revealed robust cerebellar activation in various mentalizing tasks, especially the posterior cerebellar Crus I & II (Metoki et al., 2021b; Van Overwalle et al., 2015; Van Overwalle et al., 2020). Further support for cerebellar involvement in social processes comes from various clinical studies. For example, patients with cerebellar damage display social deficits in inferring other's minds and emotions (Clausi et al., 2019; Van Overwalle et al., 2019). Additionally, people with social deficits, such as people with autism and schizophrenia, consistently showing distorted cerebellar volumes, functioning, or connections (Clausi et al., 2021; Cui et al., 2022; Siciliano & Clausi, 2020; Van Overwalle et al., 2021).
Cerebellum and sequencing learningWhat is the specific function of the cerebellum in non-motor mental processes? In general, the sequencing detection hypothesis assumes that the evolutionary older role of the cerebellum in constructing internal models of motor control, has evolved to include non-motor, purely mental processes in the form of event sequences without the involvement of overt movements and somatosensory responses (Leggio & Molinari, 2015). For example, when listening to music, people can enjoy rhythms or melodies, and anticipate what will likely happen in the upcoming notes, even they do not play themselves and do not see or imagine someone else playing.
Extending this hypothesis to the social domain, researchers propose that the cerebellum contributes to mentalizing by building internal models of repeated social sequences, and then automatizes and adjusts these models according to the current reality during social interactions (Van Overwalle et al., 2019; Van Overwalle et al., 2021). To illustrate, during daily social interactions, in some cultures we gradually learn that people we are close to, typically smile at us, stretch out their arms and hug us when we greet them. These sequential social actions are registered as an internal model for how close others greet us, and how we automatically respond. For example, we may spontaneously open our arms to hug and greet them back. If, however, they suddenly hesitate when we hug them, we register that something is different. More importantly, most of the time these behavioral sequences are implicit, automatic, and requiring little conscious monitoring. We often learn and perform these temporal patterns of social behavior implicitly and adjust our behaviors to novel social contexts implicitly.
To measure implicit learning, one well-known paradigm is the classic serial reaction time (SRT) task (Nissen & Bullemer, 1987). In this paradigm, participants rapidly respond to sequences of stimuli which are surreptitiously repeated (e.g., spatial locations on a screen). Faster responding to this hidden repetition and slower responding to any disruptions indicates successful implicit learning.
To explore this sequence detection function in a social context, Ma and colleagues designed a novel belief serial reaction time task (Belief SRT task, Fig. 1A, Ma et al., 2021) which required participants to learn a sequence that included information about others’ beliefs. Participants were asked to report what two protagonists believed (e.g., how many flowers were offered to them). To resolve the task, they had to realize that protagonists’ beliefs might converge with or differ from reality, resulting in true and false beliefs respectively. As shown in Fig. 1A, when protagonists were oriented towards the flowers, they could see the flowers and held a true belief about reality (e.g., Trial 1). Conversely, if they were oriented away from the flowers, they were not aware of any changes and therefore held a false belief (e.g., Trial 2). This is a conventional set-up in mentalizing research (Saxe et al., 2006). Crucially, unbeknownst to participants, there was a standard sequence of these true-false beliefs that was repeated over many trials (Fig. 1C). Although learning the sequence was implicit, as participants did not know the existence of the sequence beforehand and could not fully reproduce this sequence after the task, they showed learning by responding faster to repetitions of this hidden standard sequence during an intensive Training phase, and slower after any disruption in a Test phase (Ma et al., 2021). While faster responses in the Training phase reflect a general learning process, slower responses to disruptions of the specific sequence in the Test phase demonstrate sequence-specific learning.
Schematic example showing the first 6 trials of the Standard sequence in the Belief SRT task [A] and the Cognitive SRT task [B]. On each trial, participants had to report the number of flowers as seen by the protagonists (Papa Smurf or Smurfette in the Belief SRT task), or depending on the color variation of the shapes (square or circle in the Cognitive SRT task). Without being informed, belief orientations/color variations followed a Standard sequence. In the Belief SRT task, when the protagonist was oriented to the screen and could see the flowers (true trial), the number of target flowers had to be reported from the current trial; when the protagonist was oriented away from the screen and could not see the flowers (false trial), the number of target flowers had to be reported from the previous true trial from the same protagonist. Similarly, in the Cognitive SRT task, a blue square or a green circle indicated that the number of flowers had to be taken from the current trial (= true trial), while an orange square or black circle (= false trial) indicated that the number of flowers had to be taken from the previous true trial from the same shape. The number of flowers was random (1 or 2), making the response unpredictable, and sequence learning dissociated from motor responses. Each trial was self-paced, with all stimuli remaining on the screen for 3000 ms until a response was given, followed by a response-stimulus interval of 400 ms before the next trial started. [Bottom Inset] The inset shows an enlargement of the target stimulus, consisting of a pair of one or two flowers surrounded by clovers (as a distraction) of approximately the same shape and color. [Trial 1 - 2] To illustrate the instructions for the Belief SRT task, in Trial 1, there is one flower that Papa Smurf can see because he is oriented toward the screen, meaning that the correct response is 1. In Trial 2, there are two flowers. Because Papa Smurf is oriented away from the screen, he cannot see the number of flowers on this trial, hence he still holds the belief to have received one flower which he last saw on the previous (1st) trial. The correct response is thus again 1. In the Cognitive SRT task, Trial 1, there is one flower. Because the color of the square is blue, the correct response is the observed number of flowers, or 1. In Trial 1, the square is orange, so participants must report the number of flowers from the blue square from the previous (1st) trial. The correct response is thus, again, 1. [C]. The Standard sequence in the Belief SRT task. M = male (Papa Smurf), Fe = female (Smurfette), T = true, Fa = false. In the Cognitive SRT task, the sequence is identical, and stimuli were replaced by shapes and colors, as depicted in [A-B] (i.e., Male True = Blue Square; Male False = Orange Square, Female True = Green Circle, Female False = Black Circle).
A non-social Cognitive SRT task involving executive control processes was also created (Fig. 1B). Shapes replaced protagonists and colors replaced true/false beliefs, but otherwise, the structure of the task remained identical. Specifically, instead of mentalizing, colored shapes in the Cognitive SRT task required participants to respond to the current situations (= true belief) or inhibit their responses to the current situation and report the amount of flowers from previous trails (= false belief). Nonetheless, implicit Cognitive SRT learning was also demonstrated, in that participants showed general and specific learning. However, participants generally responded slower on the Cognitive than the Belief SRT task (Ma et al., 2021).
One critical aspect of the Belief and Cognitive SRT tasks is that the hidden standard sequence was unconfounded by participants’ motor responses. Responding was devoid of motor learning, because participants’ motor responses to indicate the number of flowers were randomly determined. Consequently, the Belief and Cognitive SRT tasks reflect pure perceptual learning (i.e., perceived sequences of beliefs based on orientation in the Belief task, and colors in the Cognitive task), without any aid from motor actions.
As noted earlier, previous behavioral research demonstrated implicit learning of both Belief and Cognitive sequences (Ma et al., 2021). A follow-up neuroimaging study using the same paradigm demonstrated that the posterior cerebellar Crus I was preferentially involved in the Belief SRT task, whereas more anterior cerebellar lobules VI/VIII were involved in the Cognitive SRT task when participants began to learn the hidden sequence (Ma et al., 2021). Also, when directly comparing the Belief and Cognitive tasks, the posterior cerebellar Crus II was consistently and more highly engaged in learning social sequences (Fig. 2, Ma et al., 2021). Overall, this neuroimaging study showed cerebellar involvements in Belief and Cognitive SRT tasks, but with differences in the exact location of cerebellar activation.
Sagittal views of cerebellar activations when participants learned the standard sequences. A prior neuroimaging study (Ma et al., 2021) showed that the Belief SRT task recruited more posterior cerebellar parts whereas the Cognitive SRT task recruited more anterior cerebellar parts. Moreover, higher posterior cerebellar Crus II activation was shown in the Belief than the Cognitive SRT task.
Non-invasive transcranial direct-current stimulation (tDCS) has gained considerable interest in recent years, as it can demonstrate a causal effect of the targeted brain area and specific functions, and could also be used as a potential clinical intervention to improve brain plasticity and learning. tDCS uses direct electrical currents to stimulate specific parts of the brain, by passing a low intensity current (e.g., 1 and 2 mA) through two electrodes placed over the scalp above the target brain area to stimulate it. Neural excitability is generally increased by anodal and decreased by cathodal stimulation (Bikson et al., 2016; Paulus et al., 2016). tDCS has been applied during the execution of tasks (concurrent effect), or before the execution of a particular task (post-intervention effect, van Dun et al., 2016). tDCS has been used with the classic SRT task to examine the role of the cerebellum in motor sequence learning. Studies found that, compared to sham, participants responded generally faster when given anodal cerebellar tDCS during (Liebrand et al., 2020) or after the stimulation (Ferrucci et al., 2013). More importantly, several cerebellar tDCS studies provide initial evidence that stimulating the cerebellum modulates performance of various affective and social tasks. Ferrucci et al. (2012) found that after 20 min of anodal stimulation, participants recognized negative emotions faster than before stimulation. Importantly, a recent study found that concurrent anodal cerebellar tDCS improved participants’ performance in correctly predicting social behaviors (e.g., grasping a glass for drinking versus offering to another person) under high uncertainty situations, whereas it did not improve performance in predicting non-social moving shapes (e.g., square versus rectangle; Oldrati et al., 2021). Given these promising results, in the current study, we explored the effect of anodal cerebellar tDCS on implicit social and cognitive sequence learning.
Recall that our previous neuroimaging study showed that the Belief SRT task activated the posterior cerebellum whereas the Cognitive SRT task activated the anterior cerebellum, and that most activations were located in both cerebellar hemispheres (Fig. 2, Ma et al., 2021). Therefore, we targeted the posteromedial cerebellum as this anatomical position is effective in modulating task performance in social and cognitive domains (Ferrucci et al., 2013, 2012). We also examined tDCS effects after different time intervals. Specifically, participants engaged in the Belief or Cognitive SRT tasks concurrently with stimulation, 30 min after stimulation and then again 1 week after the stimulation.
Overall, we hypothesized that the cerebellum is causally involved in sequence learning of social mentalizing and cognitive control. This should be revealed by replicating general learning and sequence-specific learning as in previous implicit Belief and Cognitive SRT research (Ma et al., 2021; Ma et al., 2021), and more importantly, by demonstrating that participants respond faster when they are given anodal cerebellar tDCS, as found in previous anodal cerebellar tDCS studies (Ferrucci et al., 2013, 2012; Liebrand et al., 2020; Oldrati et al., 2021). We also hypothesized that the closer proximity of tDCS to the posterior cerebellum would affect plasticity in this area more compared to the anterior cerebellum, and hence will favor Belief compared to Cognitive SRT learning. Moreover, we also speculated that improvements in sequence learning could be maintained over a longer period (e.g., week) after stimulation, similar to previous tDCS studies (Ferrucci et al., 2013; Firouzi et al., 2021; Savic & Meier, 2016).
MethodParticipantsA total of 138 healthy, Dutch-speaking participants were recruited. Participants were randomly assigned to receive either anodal or sham stimulation with the Belief or Cognitive SRT tasks to avoid any carry-over effects of sequence learning (Geiger et al., 2018) or ineffective blinding of brain stimulation (Jongkees et al., 2019). After the experiment, the data from thirty-two participants were excluded. Fifteen participants dropped out before the long-term post-intervention sessions. Thirteen participants had very high error rates (i.e., errors above the 3rd quartile plus 1.5 times the interquartile range, i.e., 1st vs. 3rd quartile difference). Four participants showed high awareness of sequence knowledge in the post-hoc sequence recollection test (i.e., correct recollection was above the half-length of the sequence; among them, three participants received the anodal Belief SRT task, and one participant received the sham Belief SRT task).
Hence, valid data were acquired from the remaining 106 participants (25 males, age 18-35 years, mean age 20.3±3.3). A statistical prior power analyses using G*Power3 (Faul et al., 2007) revealed that 92 participants are required in order to obtain a power of 90%, α = 0.5 with a rather small size effect (f = 0.15). For the remaining participants, 25 participants received the anodal Belief SRT task (7 males, mean age 20.3±3), 27 participants received the sham Belief SRT task (7 males, mean age 20.7±4.2), 28 participants received the anodal Cognitive SRT task (6 males, mean age 19.8±2.3), and 26 participants received the sham Cognitive SRT task (5 males, mean age 20.5±3.5). All participants gave written informed consent with the approval of the UZ Brussel Ethics Committee.
Stimuli materialThe stimulus materials are identical to those used in the previous behavioral and fMRI study on implicit sequence learning (Ma et al., 2021; Ma et al., 2021a).
Implicit belief SRT taskIn the implicit Belief SRT task (Fig. 1A), the target was 1 or 2 flowers, appearing in one of four horizontal locations, marked by four little smurfs (who offered the flowers), on the top of the screen. The target flower(s) was presented along with clovers as distractors, which occupied other remaining locations, resulting in two plants on each location. The two protagonists, Papa Smurf and Smurfette, were each shown individually at the bottom of the screen with their faces orientated either toward or away from the screen.
Participants were instructed: “One of the four little smurfs will give the flowers while Papa Smurf or Smurfette is watching (facing the screen) or not watching (facing you). Papa Smurf and Smurfette count the flowers they receive. Throughout the task, you have to track how many flowers (1 or 2) Papa Smurf or Smurfette thinks he or she will get. If they are turned with their back to the 4 smurfs, you have to indicate how many flowers they (remember that they) received last time.”
Implicit cognitive SRT taskThe following changes were made in the non-social implicit Cognitive SRT task (Fig. 1B). The target flower(s) was marked by four sidewalk boards instead of little smurfs. The orientations of Papa Smurf (true and false) and Smurfette (true and false) were replaced by squares (blue and orange) and circles (green and black) respectively. Thus, the four distinct pictures used in the implicit Belief SRT task were replaced by four distinct pictures of colored shapes in the implicit Cognitive SRT task.
Participants were instructed: “At the bottom of the screen is a square or circle. Throughout the task, you have to follow how many flowers (1 or 2) there are at the blue square or the green circle. If the square is orange, you must report the previous number of flowers from the blue square. If the circle is black, you have to report the previous number of flowers from the green circle.
In both Belief and Cognitive SRT tasks, each trial started after a response-stimulus interval of 400 ms. Responses were self-paced, and all stimuli remained on screen for 3000 ms until a response was given. When participants made a wrong response or when they did not respond within 3000 ms, the word “Error” appeared (“Fout” in Dutch) for 750 ms on the screen. After each block, participants received feedback about their mean reaction time and error rate, and they were encouraged to make less than 5% errors. Participants got a break of 15 s after every two blocks. Meanwhile, the number of flowers was randomly determined at every trial, leading to random motor responses, and a dissociation between the sequence of belief orientations/color variations and motor responses, while the sequence embedded in the two tasks and the instructions were structurally the same (Fig. 1C).
Cerebellar transcranial direct-current stimulationThis brain stimulation protocol was replicated from a previous cerebellar tDCS study (Ferrucci et al., 2013), except for the use of the tDCS equipment. A Soterix Medical 1 × 1 tDCS Low-Intensity Stimulator was used to deliver cerebellar tDCS via a pair of rectangular saline-soaked synthetic sponge (5 × 7 cm) with round rubber (50 mm diameter) electrodes inside the sponge: the active electrode was positioned horizontally on the median line 2 cm below the cerebellar inion and the reference electrode was positioned vertically over the right upper arm (Ferrucci et al., 2013) . The stimulating current was an anodal direct current at 2 mA intensity and was delivered for 20 min over the posteromedial cerebellum. As noted earlier, we stimulated the posteromedial cerebellum as in Ferrucci et al. (2013) to target the bilateral cerebellum, where we found activation in our previous fMRI study (Ma et al., 2021, Fig. 2).
For the anodal stimulation, the current intensity was slowly ramped up from 0 to 2 mA in 30 s, then this current intensity was maintained for 20 min, and then ramped down from 2 mA to 0 in 30 s. For the sham stimulation, the current intensity was slowly ramped up from 0 to 2 mA and then slowly ramped down to 0 mA in one minute. After 20 min, this slow ramping-up and ramping-down of the current intensity was applied again.
We used a self-report questionnaire to assess side effects due to tDCS intervention (Brunoni et al., 2011), which included 10 most common complaints (headache, neck pain, scalp pain, tingling, itching, burning sensation, skin redness, sleepiness, trouble concentration, acute mood change). Responses on each item require participants to indicate “Do you experience any of the following symptoms or side-effects?” on a 4-point scale (1 = absent, 2 = mild, 3 = moderate, 4 = severe).
Experiment procedureThe participants were blind to the type of tDCS delivered (sham versus anodal), however, blinding the experimental researcher was impossible with the current device. So, we used a single blind design. Note that since the reference electrode was placed over participants’ right upper arm, they were required to complete the task using their non-dominant left-hand.
Concurrent sessionIn the concurrent session, participants first completed a number of practice trials to familiarize themselves with the task. This consisted of two blocks with 24 trials each and these practice data were excluded from further analysis. Next, they completed a number of baseline trials. This also consisted of two blocks with 24 trials each. These trials were recorded to measure participants’ baseline performance before the main task. The sequence of the trials in these practice and baseline blocks was different than in the main experiment.
After the practice and baseline trials, participants started the Belief or Cognitive SRT task (Fig. 3B), which consisted of 11 blocks with 48 trials each. The full version was divided into a Training and a Test phase. In the initial Training phase, the Standard sequence was repeated throughout 5 blocks (Standard blocks 1–5). This Standard sequence consisted of 16-trials of varying Protagonists (smurfs / shapes) and Orientations (beliefs / colors; Fig. 1C) and was repeated three times per block. In the Test phase, Blocks 6, 8, 9, and 11 were identical to the Standard blocks in the Training phase. In Blocks 7 and 10, the Standard blocks were inconspicuously replaced by Random blocks. There were two types of Random blocks. In a Total Random block, Protagonist (smurfs / shapes) and Orientation (beliefs / colors) were totally randomized with the limitation of at most 2 subsequent trials of the same Orientation, consistent with the Standard blocks. In a Random Orientation block, only Orientation (beliefs / colors) was changed into a different pseudo-random sequence while Protagonists (smurfs / shapes) remained identical as in the Standard blocks (See Supplementary Table S1). The order of the Total and Orientation Random blocks in Blocks 7 and 10 was counterbalanced between participants.
[A] Schematic representation of the experimental design. [B] The SRT task with block numbers 1-11. S = Standard block (with standard sequence). R = Random block. Note that every block has a length of 48 trials. One random sequence was created for Protagonist and Orientation together (i.e., Total Random), and one pseudo-random sequence was created to test the learning of Orientation (i.e., Random Orientation). Participants would perform one Total Random and one Random Orientation block in the Test phases, and the order of two Random blocks was counterbalanced among participants.
While performing the SRT task, participants received either anodal or sham stimulation for 20 min, regardless of the duration participants spent on completing the SRT task (Fig. 3A). In the current study, participants received stimulation after completing the task for an average of 4.27 min.
Short-term post-interventionAfter tDCS, participants took a break for 30 min (Fig. 3A). During this break, participants were asked to finish a questionnaire that assessed potential adverse effects associated with brain stimulation (Brunoni et al., 2011). After completing the questionnaire, participants were allowed to do anything they wanted as long as they would rest and not engage in anything cognitively or socially taxing. They could stay in the lab or have a short walk outside. Coming back from the 30 min break, participants completed a short version of the Belief/Cognitive SRT task which only consisted of Test phase, identical to the one in the full version (Fig. 3B).
Long-term post-interventionThe long-term post-intervention took place one week later (Fig. 3A). In this session, participants first completed two practice blocks to help them to re-familiarize with the task. Then, the same, full version of the Belief/Cognitive SRT task was performed again without the application of tDCS (Fig. 3B). After the task, an online post questionnaire was completed to test whether participants became aware of the sequence. Participants were first asked “whether you notice something particular or not”. Next, they were asked to reproduce, as accurately as possible, the "order in which Papa Smurf and Smurfette appeared" and whether or not they "could or could not see who gave the flowers” in the Belief SRT task; and the “order in which squares and circles appeared” for each combination of shape and color in the Cognitive SRT task. Sequence retrieval accuracy was scored by taking the maximum length of a correct sequence, starting at every possible point in the sequence.
Statistical analysis for sequence learning and stimulation effectsParticipants’ number of errors and mean reaction times (RTs) per block were analyzed. For the RTs analysis, responses during and immediately after an error were excluded. In addition, each participant was given a sequence awareness score ranging from zero to sixteen, based on their longest correct sequence recollected in the post-check of the final, long-term post-intervention session. This recollection score was used as a covariate in the following mixed ANOVA analyses on learning effects to minimize the effect of any potential sequence knowledge.
First, a general learning effect during the Training phase was tested by a mixed ANOVA with Standard Blocks (Block 1 to 5) and Sessions (Concurrent and 1-week post) as within-participants factors, and Task (Belief versus Cognitive) and Stimulation (Anodal versus Sham) as between-participants factor. This will not be applicable to the short-term post-intervention as it only included the Test phases.
Second, sequence-specific learning effects were tested by a mixed ANOVA with Block Type (Random versus Standard Blocks [the average of Standard Blocks adjacent to each Random block) and Session (Concurrent, 30-mins post and 1-week post) as within-participants factors, and Task (Belief and Cognitive) and Stimulation (Anodal versus Sham) as between-participants factors. Given that we have two types of Random blocks (i.e., Total Random and Random Orientation block; i.e., Block 7 or 10) and different Standard Blocks adjacent to them (i.e., Blocks 6–8 or 9–11 respectively), these two types were tested separately.
Significant main and interaction effects were further tested by a post hoc Bonferroni test. The significance level was set to 0.05, and two-tailed tests were applied. When the sphericity assumption was violated for ANOVA, the Greenhouse-Geisser correction is reported.
A Bayes factor analysis was also used to evaluate the effects found in the experiment (JASP0.16.0.0). Here we relied on Bayes factors (BF10) for interpreting our main results. BF10 indicates the strength of the evidence in favor of alternative hypothesis (H1) over the null hypothesis (H0). For example, if BF10 = x, this means that the data are x times more likely under the alternative hypothesis (e.g., there is a learning effects) than under the null hypothesis (e.g., there is no learning effect). Results with 1 < BF10 < 3 indicate anecdotal evidence to support the alternative hypothesis, BF10 > 3 indicate substantial evidence to support the alternative hypothesis, and BF10 > 10 indicate strong evidence to support the alternative hypothesis (van Doorn et al., 2021).
ResultsManipulation check: limit awareness of sequence and stimulationAwareness of the (repetition of the) Standard sequence was assessed at the end of the long-term post-intervention. Participants were first asked to answer a sequence awareness question (“whether you notice something particular or not”), and then had to reproduce the whole sequence for the Protagonists (Smurfs / Shapes) and their Orientation (Beliefs / Colors).
Most participants (57%) reported no sequence awareness, and the number of these participants was comparable across Task and Stimulation by chi-square tests (all ps > 0.3). To exhaustively test awareness, each participant was given a sequence awareness score ranging from zero to sixteen, based on their longest correct sequence recollection. The mean recollection score was 2.87 out of 16, indicating a limited sequence awareness in the current study. A two-way ANOVA showed that the recollection score did not differ between Task (Belief versus Cognitive) and Stimulation (Anodal versus Sham, all ps > 0.1, M ± SD: Anodal Belief: 3.24 ± 1.3; Sham Belief: 2.74 ± 1.6; Anodal Cognitive: 2.89 ± 1.5; Sham Cognitive: 2.62 ± 1.7 see details in Supplementary Table S2). To minimize the effect of any potential sequence knowledge, this recollection score was used as a covariate in the mixed ANOVA analyses on learning performance (see below), and results showed this covariate was insignificant in these analyses.
Potential side effects due to tDCS were assessed through a self-report questionnaire (Brunoni et al., 2011). Consistent with previous studies, none of the participants reported major side effects and the most common complaints were itching and skin redness under the electrodes (Brunoni et al., 2011; Jongkees et al., 2019). A two-way ANOVA showed that there were no differences between Task and Stimulation (ps > 0.1), except that participants reported more itching and skin redness under anodal stimulation than sham (see details in Supplementary Table S3). Because almost all participants reported that this was the first time they participated in a tDCS study (only four participants had tDCS experience before), and because we used a between-participant design, it is unlikely that participants were aware of the distinct Stimulation types only based on these subjective experiences of the tDCS side effects. Therefore, the experienced side effects most likely did not modulate or confound the learning effects reported below.
Baseline measurement showed no task differences before stimulationA 2 Stimulation (Anodal versus Sham) × 2 Tasks (Belief versus Cognitive) ANOVA was conducted to examine baseline performance before the main SRT task at the concurrent session (2 baseline blocks). Results revealed no significant difference in RTs or errors at baseline (all ps > 0.2, see details in Supplementary Table S4).
Anodal stimulation accelerated RT performance on the cognitive but not the Belief SRT tasksTo examine the influence of anodal tDCS, mixed ANOVAs were applied on RTs and errors. Here, we first report the results for RTs. As noted earlier, these analyses were conducted with awareness (i.e., recollection score) as a covariate, but the results showed no substantial effect of this covariate and were, therefore, omitted (but are accessible, see Data Availability).
Response times and general learningA mixed ANOVA was used with five Standard Blocks in the Training phase (Blocks 1–5) and Session (Concurrent and 1-week post) as within-participants factors and Task (Belief versus Cognitive) and Stimulation (Anodal versus Sham) as between-participants factors. As shown in Fig. 4A, participants responded faster across Standard Blocks during Training, revealed by a significant main effect of Blocks 1-5 (F(3.57, 360.85) = 10.67, p < 0.001, η2 = 0.10, BF10 = 80577). Compared to the concurrent session, participants also responded faster in the 1-week post intervention, shown by a significant main effect of Sessions (F(1, 101) = 122.16, p < 0.001, η2 = 0.55, BF10 = 2.16E+242, MD1-week post-Concurrent = -270 ms).
Mean RTs [A] and errors [B] after anodal and sham stimulation for the Belief and Cognitive SRT tasks. “*” indicated significant RT differences in general learning and sequence-specific learning; “+” indicated significant RT differences between anodal and sham stimulation (uncorrected for multiple comparisons). S = Standard Block, TR = Total Random block, RO = Random Orientation block. Error bars = Standard errors of the mean. Random blocks of the same type and their adjacent sequence blocks were collapsed in the analysis.
There was a main effect of Task (F(1, 101) = 5.08, p = 0.03, η2 = 0.05, BF10 =1, MDBelief-Cognitive= -52 ms), of Stimulation (F(1, 101) = 6.60, p = 0.01, η2 = 0.06, BF10 = 3, MDAnodal-Sham = -59 ms), and an interaction between Tasks and Stimulation (F(1, 101) = 5.09, p = 0.03, η2 = 0.03, BF10 = 3). Post-hoc comparisons with Bonferroni correction showed faster responses in the Anodal than Sham condition of the Cognitive SRT task (MDAnodal-Sham = -111 ms, t = -3.45, p = 0.005), but not in the Belief SRT tasks (MDAnodal-Sham = -8 ms). There was no interaction between Stimulation and Blocks (1-5), indicating that anodal stimulation did not increase further the amount of general learning. There were no other significant effects.
Response times and sequence-specific learningTwo mixed ANOVAs with Block Type (Total Random/Random Orientation versus the average of adjacent Standard Blocks) and Session (Concurrent, 30-mins post and 1-week post) as within-participants factors and Task (Belief and Cognitive) and Stimulation (Anodal versus Sham) as between-participants factors was applied on RTs (Fig. 4A).
The analysis on Total Random Blocks showed a main effect of Block Type which revealed sequence-specific learning by slower responses in the Total Random versus Standard blocks (F(1, 101) = 22.47, p < 0.001, η2 = 0.18; BF10 = 8.25E+09), and a main effect of Session (F(1.74, 176.07) = 36.76, p < 0.001, η2 = 0.27; BF10 = 3.414E+66). Post-hoc Bonferroni tests showed faster RTs across sessions (MD30-mins post-Concurrent = -96 ms, t = -11.48, p < 0.001; MD1-week post-Concurrent = -145 ms, t = -17.38, p < 0.001; MD1-week post-30-mins post = -49 ms, t = -5.92, p < 0.001). Participants responded significantly faster under Anodal than Sham Stimulations (F(1, 101) = 6.63, p = 0.01, η2 = 0.06, BF10 = 3) and their response times trended towards being slightly faster in the Belief than the Cognitive Tasks (F(1, 101) = 2.8, p = 0.10, η2 = 0.03, BF10 = 0.5). There was an interaction between Task and Stimulation (F(1, 101) = 4.06, p = 0.05, η2 = 0.04, BF10 = 2), and post-hoc comparisons showed that the effect of stimulation on RT was significant for the Cognitive SRT task (MDAnodal-Sham = -82 ms, t = -3.29, p = 0.008) but not for the Belief SRT task (MDAnodal-Sham = -10 ms).
The analysis on Random Orientation Blocks revealed similar results. There was a main effect of Block Type which revealed sequence-specific learning by slower responses in the Random Orientation versus Standard blocks (F(1, 101) = 13.90, p < 0.001, η2 = 0.12; BF10 = 151516), and a main effect of Session (F(1.85, 186.61) = 19.48, p < 0.001, η2 = 0.16; BF10 = 1.15E+78). Post-hoc Bonferroni tests showed faster RTs across sessions (MD30-mins post-Concurrent = -113 ms, t = -13.21, p < 0.001; MD1-week post-Concurrent = -163 ms, t = -18.96, p < 0.001; MD1-week post-30-mins post = -49 ms, t = -5.76, p < 0.001). Participants responded significantly faster under Anodal than Sham Stimulations (F(1, 101) = 7.15, p = 0.009, η2 = 0.07, BF10 = 3) and their response times were faster in the Belief than the Cognitive Tasks (F(1, 101) = 4.5, p = 0.04, η2 = 0.04, BF10 = 1).There was an interaction between Task and Stimulation (F(1, 101) = 7.28, p = 0.008, η2 = 0.07, BF10 = 4) and post-hoc comparisons showed that a stimulation effect on RT for the Cognitive SRT task (MDAnodal-Sham = -97 ms, t = -3.84, p = 0.001) but not for the Belief SRT task (MDAnodal-Sham = -0.1 ms).
Crucially, there were no interactions between Stimulation and Block Type for the two types of Random blocks, indicating the anodal stimulation did not improve the amount of sequence-specific effect in Total Random or Random Orientation blocks.
The results of the mixed ANOVAs demonstrate that participants responded faster under anodal compared to sham stimulation during the Cognitive SRT task. To further test the effect of anodal stimulation, independent t-tests were applied for each block. The results showed that RT improvements during the Cognitive SRT task appeared for most of the standard blocks in the concurrent stimulation session, and for all types of blocks in the 30-mins post and 1-week post sessions (Fig. 4A). Looking at the Cognitive SRT task more closely, we also computed a difference score between Standard Blocks 1 and 5 in the Training phases to reflect the amount of general learning, and a difference score between Random and adjacent Standard Blocks to reflect the amount of sequence-specific learning. Larger RT difference scores reflect stronger learning effects during the Cognitive SRT task. As shown in Fig. 5, although these effects were non-significant, anodal stimulation seems to show stronger sequence learning.
Cognitive SRT task: Mean RTs differences between Standard Block 1 to 5 denoted as S(B1-B5), mean RT differences between Total Random and its adjacent Standard Blocks (TR), and mean RT differences between Random Orientation and its adjacent Standard Blocks (RO). Statistical analyses showed that the improvement given anodal stimulation at the concurrent and 30-mins and 1-week post-intervention sessions was larger than the sham but nonsignificant. Error bars = Standard errors of the mean.
We also ran the same sets of mixed ANOVAs for the number of errors. The results showed that participants made more errors in the Cognitive than the Belief SRT tasks during the Training phase in the concurrent session (MDCognitive-Belief = 0.6, Fig. 4B). Also, they made more errors in Random Orientation blocks compared to adjacent Standard blocks (MDRandom Orientation-Standard = 0.9, Fig. 4B). No other learning effects were found. As these results did not appreciably change the interpretation of the learning and stimulation effects based on RTs discussed above, they are reported in the Supplementary Materials.
SummaryOverall, as we hypothesized, we found general learning and sequence-specific learning effects for both Belief and Cognitive SRT tasks across sessions. Contrary to our hypothesis, the anodal stimulation accelerated participants’ responses only in the Cognitive SRT task, and this improvement was significant during the concurrent and two post-intervention sessions. However, the anodal stimulation did not significantly improve the amount of sequence learning. There was no effect of anodal stimulation on the Belief SRT task.
DiscussionThe current study investigated the causal impact of the cerebellum on implicit belief and cognitive sequence learning. To investigate this, we stimulated the posteromedial cerebellum using anodal tDCS to see its influence on performance on a social Belief and a non-social Cognitive SRT task. Recall that by stimulating the posteromedial cerebellum, we expected to obtain tDCS effects on both Belief and Cognitive SRT learning. We also hypothesized to find somewhat stronger effects on Belief SRT learning because stimulation is closer to the posterior cerebellum involved in social mentalizing as opposed to the anterior cerebellum involved in cognitive sequence learning. Our results only partly confirm our hypothesis. We found that anodal cerebellar stimulation accelerates response time in the non-social Cognitive SRT task, although acceleration was general and not specific for the standard sequence. Importantly, we found that this RT acceleration was still present 30 min and one week after stimulation. However, contrary to our hypothesis, anodal tDCS did not accelerate responding in the social Belief SRT task. Overall, our results suggest that anodal tDCS on the posteromedial cerebellum has a positive effect on general performance in the Cognitive SRT task, but not in the social Belief SRT task.
Anodal cerebellar tDCS does not accelerate performance in the Belief SRT taskThe current results demonstrated general learning and sequence-specific learning of the standard sequences as reflected in faster RTs to the standard sequence and slower RTs to random sequences, which replicates results of previous Belief and Cognitive SRT studies (Ma et al., 2021). However, the lack of improvement after anodal stimulation on RTs in the Belief SRT task, while the RTs improved on the Cognitive SRT task, is unexpected.
One potential factor for this differential tDCS effect on type of task is familiarity and effort expenditure. When a task is familiar and does not require much mental effort, it might profit less from any attempts for further improvement, such as non-invasive neurostimulation (Bongaerts et al., 2020; Pope & Miall, 2012). To illustrate, Pope & Miall (2012) found that a complex cognitive task showed improved performance after tDCS, while an simple version of the same task did not. Similarly, a recent study showed that anodal cerebellar tDCS improved accuracy to recognize a sequence of three letters, but did not for a much simpler sequence of one letter (Maldonado & Bernard, 2021). Likewise, Ferrucci et al. (2012) found that participants recognized negative emotions faster after receiving anodal cerebellar tDCS compared to before stimulation (baseline), but showed no improvement in recognizing positive and neutral emotions. In their study, participants took longer to identify negative emotions at baseline, which may reflect a general difficulty in discriminating negative emotions from the start, which left enough room for further improvement as a result of stimulation.
How might familiarity and mental effort have impacted on our results? Evidently, our Belief SRT task involves a very familiar process of belief understanding, whereas the Cognitive SRT tasks rests on artificial and unfamiliar rules. This has been attested by previous experiments (Ma et al., 2021), which showed generally faster responses on Belief than Cognitive SRT tasks. Likewise, in the current study, there was no RT difference between Belief and Cognitive SRT tasks during the baseline, but participants adjusted quicker to the Belief SRT task. This suggests that healthy participants are quite familiar with social schemata and have extensive real-life practice with mentalizing (in the Beliefs SRT task), whereas thinking of colorful objects (in the Cognitive SRT task) is purely artificial and peculiar and rarely practiced outside the laboratory (Callejas et al., 2011; Cohen & German, 2010; Ma et al., 2021). Familiarity suggests that a process could be automatized, so that more attention can be be allocated and maintained to the repeated sequence during the whole SRT experiment. This gives less room for further RT improvement on the Belief than the Cogntive SRT task.
An alternative, but related explanation is that perhaps some neurological effects were produced by tDCS stimulation, but were not transformed into observable behavioral effects. This may be especially the case when the task is familiar and easy. To illustrate, in a concurrent tDCS and fMRI study, D'Mello et al. (2017) found that anodal tDCS successfully increased activation in the right posterior cerebellar Crus I & II when reading sentences, although no behavioral effects were observed (i.e., faster responses in sentence completion).
Together, our and previous findings suggest that cerebellar stimulation may not facilitate familiar processes such as inferring other's minds under very structured lab conditions. However, under more taxing everyday social circumstances or for clinical populations that struggle with social mentalizing, stimulating the cerebellum may provide further benefits. (Van Overwalle et al., 2021). Recent clinical studies have demonstrated that patients with autism or with cerebellar damage show social deficits in understanding others’ minds or emotions (Clausi et al., 2021; Van Overwalle et al., 2019), and are therefore prime candidates for such an approach.
Anodal cerebellar tDCS improves general performance on the cognitive SRT taskAs hypothesized, participants generally responded faster during anodal cerebellar tDCS in the Cognitive SRT task, and even after stimulation up to one week later. Fig. 4A indeed shows that RT performance on the Cognitive task was closer to performance on the Belief SRT task with anodal stimulation, which supports our reasoning that improvement was more likely to be observed in the unfamiliar Cognitive task.
There are two potential explanations for the prolonged stimulation effect. It may indicate that concurrent tDCS (i.e., during learning) can improve general performance, and this improvement could still be present and observed one week later. Alternatively, it may indicate that cerebellar excitability may have lasted one week after stimulation, and that this extended excitability caused improved performance one week later. To test these potential explanations, future studies can ask participants to perform new sequencing tasks after the intervention, and any improved performance of the novel tasks may support the idea of extended excitability. Nevertheless, the current results suggest that cerebellar tDCS benefits could extend over a longer period (at least up to one week), which is critical for potential clinical applications. This information is also useful for determining a sufficient wash-out period for future neuro-stimulation studies.
Previous work showed that participants responded faster on motor sequence learning tasks when they received anodal cerebellar tDCS (Jongkees et al., 2019; Liebrand et al., 2020; Shimizu et al., 2017). The current Cognitive SRT task extended this RT benefit to perceptual learning which was unconfound by motor responses (i.e., the sequence of shapes’ colors was not related to the answers on the number of the flowers). This is consistent with previous studies demonstrating that perceptual sequence knowledge could be obtained without help from sequential motor responses (Coomans et al., 2012; Deroost & Coomans, 2018; Deroost et al., 2009; Firouzi et al., 2021). More importantly, our result supports the idea that the cerebellum is causally involved in non-motor cognitive processes, and can be modulated by brain stimulation.
Limitation and theoretical implicationsBased on the current findings, there are several limitations and theoretical implications which are important for future research. When we delivered anodal tDCS on healthy participants, we found RT improvement on the Cognitive, but not on the Belief SRT task. As noted earlier, one potential factor influencing tDCS effects is the type of task, because it is less likely that participants benefit from stimulation when a task itself is already automatized and does not require much mental effort (Bongaerts et al., 2020; Pope & Miall, 2012). However, the current study used a between-participant design, which makes it difficult to directly compare how individual levels of effort may have impacted performance on the Belief and Cognitive tasks. For future neurostimulation studies, researchers should consider a within-participant design, which controls for individual differences. Also, as noted before, the current study suggests that brain stimulation may not lead to detectable changes in RTs or accuracy due to daily exposure, experience and automatization of social mentalizing (Oldrati et al., 2021; Pope & Miall, 2012). Therefore, researchers should be cautious about drawing conclusions that brain stimulation does not modulate performance, especially in studies using healthy participants.
The current study used protocols focusing on the posteromedial cerebellum (Ferrucci et al., 2013, 2012). It is possible that the current tDCS protocol stimulated an extensive part of the bilateral cerebellum from posterior to anterior parts. Previous studies used varying protocols (e.g., varying in intensity, montage sizes and locations) on different social tasks and found contradictory effects (Bongaerts et al., 2022; D'Mello et al., 2017; Ferrucci et al., 2012; Oldrati et al., 2021). Meta-analytic studies are needed to find a limited set of effective cerebellar tDCS protocols for a diversity of social tasks that are meaningful for theoretical insight on social cognition or practical use in everyday life. One potential avenue for future research is more focal stimulation by using high definition tDCS with multichannel optimized montages to selectively stimulate only the posterior cerebellum.
The current study showed that anodal tDCS facilitated response times. These findings are congruent with the known role of the cerebellum in learning and automatizing skills by building internal models of sequential information (Leggio & Molinari, 2015; Van Overwalle et al., 2019). However, the cerebellum does not work alone to compute this information. One dynamic causal modellng study showed that the posterior cerebellum is connected with key cerebral mentalizing areas, such as the bilateral temporoparietal junction via closed loops, during implicitly belief sequence learning (Ma et al., 2022). This suggests that the cerebellum receives social input signals from the cerebral cortex and returns feedback signals to the same brain areas. Consequently, anodal cerebellar tDCS may not only modulate the cerebellum, but also broader brain networks that the cerebellum interconnects to, resulting in stronger output signals being sent to the cerebral cortex (Pope & Miall, 2012; Rice, et al., 2021). By combining tDCS and fMRI or event-related potential (ERP)techniques, future studies could further investigate potential changes in neural signals within the cerebellum, corresponding cerebral mentalizing regions, as well as cerebello-cerebral connectivity.
During implicit sequence learning, it is difficult to find a single point to indicate participants’ shift from being unaware to being aware of the sequence (Ferrucci et al., 2013). As awareness of sequence knowledge is a continuum, we controlled its influence by using the length of sequence recollection as a covariate in the ANOVAs. Interestingly, in a recent study, healthy participants who were informed of the existence of a standard sequence, showed better performance in recalling the sequence after anodal cerebellar tDCS than sham (Liebrand et al., 2020). This is also the case in the current study. Even when participants did not demonstrate changes in belief sequence learning during and after receiving anodal stimulation, they did slightly better (at least numerically) in the post-check when they were asked to recall the standard belief sequence. One potential explanation is that participants who received anodal stimulation can recall the sequence better, because anodal stimulation may have freed up more resources to pay attention to, store and retrieve this knowledge more solidly. As this better performance on recall was not statistically significant in the current study, these results should be interpreted with caution. To further address this, future neurostimulation studies could use explicit instructions that inform participants about the social sequence, to investigate whether tDCS stimulation under these circumstances can improve any learning performance, including mean length of the correctly recalled SRT sequence.
ConclusionThe current study showed that anodal tDCS on the cerebellum improved general performance on Cognitive sequence learning of healthy young adults, while Belief sequence learning which was structurally identical, remained unaffected. This supports the idea that the cerebellum is involved in learning sequences, although in a different manner than we had hypothesized. One potential explanation for the lack of improvement on our social Belief SRT task is that social mentalizing is a very familiar and automatized process for healthy participants, so that more attention and mental effort is allocated to the novel sequence during the task, and hence leaves little room for further improvement. Future studies are needed to find a set of suitable protocols for targeting the posterior cerebellum involved in social processes, including the use of clinical populations with limited social capacities, which may potentially further increase implicit social sequence performance. Studies should also investigate whether or how cerebellar stimulation modulates corresponding cerebral areas or cerebello-cerebral connectivity.
Data availabilityAll requested (pseudonymized or anonymous) data are available upon request, excluding data that allow identifying individual participants. Belief and Cognitive SRT tasks are also available upon request. Results of all analyses, including auxiliary analyses not reported in detail in this article, can be accessed via the Open Science Framework: https://osf.io/mn2yq/?view_only=8b05cfdc6d6a4730a02a47b74754d5a2.
This research was funded by SRP57 awarded by the Vrije Universiteit Brussel to Frank Van Overwalle, and a CSC fellowship awarded to Qianying Ma.